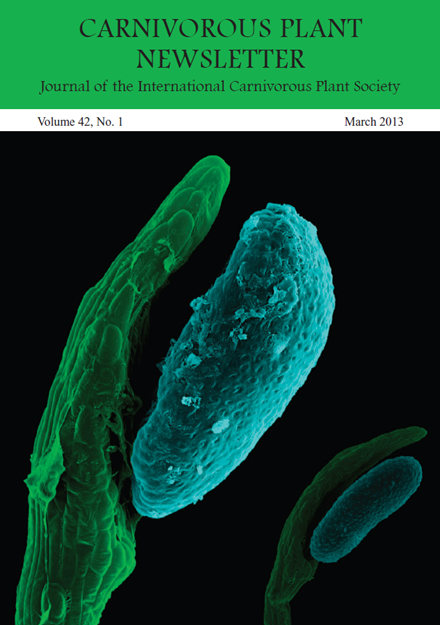
Technical Refereed Contribution
Catapults
into a deadly trap: The unique prey capture mechanism of Drosera glanduligera
Siegfried R.
H. Hartmeyer • DE-79576 Weil am
Rhein • Germany
Irmgard Hartmeyer • DE-79576 Weil am Rhein • Germany
Tom Masselter
• Plant
Biomechanics Group Freiburg • Botanic Garden • Faculty of Biology •
University of Freiburg • Schänzlestrasse 1 • DE-79104 Freiburg im Breisgau • Germany
Robin Seidel • Plant Biomechanics Group Freiburg • Botanic Garden • Faculty of Biology • University of Freiburg • Schänzlestrasse 1 • DE-79104 Freiburg im Breisgau • Germany
Thomas Speck • Plant Biomechanics Group Freiburg • Botanic Garden • Faculty of
Biology • University of
Freiburg • Schänzlestrasse 1
• DE-79104 Freiburg
im Breisgau • Germany
Simon Poppinga • Plant Biomechanics Group Freiburg • Botanic Garden • Faculty of
Biology • University of
Freiburg • Schänzlestrasse 1
• DE-79104 Freiburg
im Breisgau • Germany
Keywords: catapult-flypaper-trap,
cultivation, Drosera glanduligera, functional morphology, plant
biomechanics, snap-tentacles.
Cover picture: Colorized SEM images of terminal discs and raised heads of Drosera glanduligera snap-tentacles. Image by the Plant
Biomechanics Group Freiburg and I. & S. Hartmeyer.
Introduction
Active
trapping mechanisms constitute some of the most spectacular examples
for how carnivorous plants catch their prey (Darwin 1875; Lloyd 1942;
Juniper et al. 1989). Recently, we showed that the Pimpernel Sundew (Drosera glanduligera Lehm.) possesses active combined catapult-flypapertraps which work with a sophisticated two-step mechanism (Poppinga et al.
2012): after mechanical stimulation, elongated marginal snap-tentacles
at the trap periphery rapidly fling the prey, often with its dorsal
side first, onto sticky glue-tentacles on the leaf blade within less
than 1/10 second. Subsequently, stimulated mechanically by the impact,
slower glue-tentacles lift the prey into a deeply concave leaf-center
where digestion takes place. The snap-tentacles have been analyzed in
respect to their kinematics, functional morphology and anatomy, and our
observations confirm a complex adaptation to carnivory. From the very
beginning we intended to accompany our research with informative
documentaries (Hartmeyer & Hartmeyer 2012a,b) and to provide
this additional article to the readers of the CPN. It features a
brief summary of the main results, some extended background
information, further original morphological observations, and
interpretations (surely featuring issues to discuss) as well as a
detailed description of how to cultivate this sophisticated carnivorous
plant.
Background Story
Remarkably, the rapid snap-tentacle
motion of the Pimpernel Sundew has not been noticed for a long time, ranging
for more than 150 years from the species description (Lehmann 1844) until the
end of the last millennium. Even in the otherwise comprehensive benchmark books
on Australian carnivorous plants published more than one hundred years after
the first species description, this mechanism was not mentioned (Erickson 1968;
Lowrie 1989). The same holds for the article of Seine & Barthlott (1993)
who provided a detailed comparative anatomical survey of the apical tentacle
parts of numerous Drosera species and
described D.
glanduligera to possess bilaterally symmetric tentacles with a raised head, a feature
that is unique in the genus. The first person to report on snap-tentacle action
was Richard Davion who published two important field reports in “Flytrap News”,
the newsletter of the Carnivorous Plant Society of New South Wales (Davion
1995; 1999), mentioning that “… the dry pads [of the Pimpernel Sundew] are quite
able to flick ants into the center of the traps.” He noticed the fast snap-tentacle
motion of D.
glanduligera already in 1974 at Cannington Swamp near Perth as a 9-year-old boy
(pers. comm.). In 2003, Davion contacted Irmgard and Siegfried Hartmeyer and provided
seeds with the request to examine and confirm the rapid motion, which was
successfully accomplished and published in Das Taublatt, the journal of the
German CPS (GFP) (Hartmeyer & Hartmeyer 2005). In addition, a video
documentary (Hartmeyer & Hartmeyer 2006) with detailed macro-shots was
released and presented at the 2008 ICPS conference in Frostburg, constituting a
comparative morphology of the multifold elongated marginal tentacles in the
genus Drosera and also including
first speed measurements (see also a contribution in McPherson 2008). An
upgraded article in the CPN on the snap-tentacle phenomenon followed in 2010
(Hartmeyer & Hartmeyer 2010). The fast tentacle motion performed by a Drosera species drew the
attention of plant biologists who work on “rapid plants” on the otherwise
rather inconspicuous Pimpernel Sundew. However, Davion’s assertion that prey
can be thrown into the leaf-center became meanwhile adopted by several authors
(Gibson & Waller 2009; Bourke & Nunn 2012), but was never confirmed by
prey capture experiments or scientifically conducted observations in the field.
In January 2012 we decided to bridge this knowledge gap by experimentally
feeding cultivated D. glanduligera plants to record the trapping motion
and furthermore to conduct morphological and anatomical investigations.
Cultivation
of Drosera
glanduligera
The Pimpernel Sundew is an annual winter grower with a wide distribution
range across the southern regions of Australia (Erickson 1968).
Therefore it needs cool nights but warm and bright days to thrive well. After
cultivating the plants for almost ten years in the Northern Hemisphere, our
observations show that the germination of D. glanduligera seeds is triggered
when the night temperature drops significantly below 8°-10°C for approximately
3-7 days after the seeds have been sown on a standard peat-sand-mixture (partly
containing also pumice gravel or Perlite) in June, and remain on the wet soil in
full sunlight during the summer. A reduced “hot season” may avoid germination
in time and cause a delay for a whole year (see below). Due to decreasing night
temperatures in autumn, germination usually starts in October in the
south-western region of Germany. In 2012, the first seeds sown in early June
germinated after only three cool nights (4°-5°C) in late September. We do not
use any additional treatments to improve the germination such as smoked water,
gibberellic acid, or other methods and substances, respectively. For the
experiments described, approximately 300 seeds were sown in mid-July 2010, from which about 200
germinated with a surprising extreme delay in October 2011, and from which
pproximately 150 plants matured. In order to thrive well, the temperature after
germination has to remain only slightly above 0°C at night until the beginning
of March. During the daytime, plenty of light and temperatures of up to 15°-25°C
are necessary. In January 2012, the temperature for our test plants ranged
between 0.8° and 27°C. An electric frost protection unit avoided a cooling down
below the freezing point, and during the day we achieved ideal conditions with
a sunny south-western exposure position, combined with a 400 watt metal halide
lamp. If the night temperature rises above 8°C before March, premature
flowering is triggered, resulting in early plant death and a reduced seed
production. Apart from this, another factor proved to be extremely important
for the plants: in addition to the correct light and temperature conditions the
plants need constant nutrition supply from the very beginning. Only then the
seedlings metamorphose, in the first 4-6 weeks of initial growth, from the
juvenile phase with simple sticky traps to the adult phase with catapult-trap
leaves. Collembola
(springtails) most
likely constitute the main natural prey for D. glanduligera and other
Australian sundew species (Verbeek & Boasson 1993; Watson et al. 1982). Ideally,
if one has living springtails in the plant pots and soil they will be successfully
caught and digested (Hartmeyer & Hartmeyer 2010). Otherwise, the feeding
necessary for D. glanduligera is quite time-consuming and takes
place in several consecutive phases, depending on the age and size of the
plants. We use standard fish food flakes which are comminuted between the
fingers. The smallest pieces are picked up using a forceps (best with magnifier
glass) to feed the plants leaf by leaf. This procedure is performed for plants
of about 2-5 mm in diameter. In the next phase, when the leaves are about 3 mm
in diameter, we feed them with fruit-flies that are commercially available in
garden centers or pet shops. The flies are cut into appropriate pieces (considering
the small leaves) using a scalpel. This provides ideal nutrition to the plants
as early as possible, avoids over-feeding them, and reduces the risk of mold
formation. Later, when the leaves are about 5-6 mm in diameter, there is no
major risk in feeding even 2-3 fruit-flies at a time per leaf. New leaves are
reproduced every 3-4 days until the end of the growing season. Therefore, the
feeding should be repeated about twice per week to achieve permanent growth.
Flowering, Seed Production, and Seed
Morphology
With the above mentioned conditions,
flowering was observed to take place from early March until late April 2012
(Fig. 1a). When the flowers are open, night temperature may rise to 10°-12°C
and the day temperature may exceed even 30°C without any visible deterioration
of the plants. Providing as much light as possible is highly recommended. In
cultivation the plants are mostly self-pollinating and multiple seed-pods will
emerge on the inflorescence stalk, while new flowers are still produced on the
stalk apex (Fig. 1b). In May 2012 we observed most of the seed-pods to ripen.
At the same time, the rosettes started to become brownish and the plants died
back within a few days. By end of May to early June 2012 we harvested the
seeds. However, it is actually quite tedious to free them from dried plant
matter, sticking to the still gluey seed pods. Each plant can produce several
hundred seeds. Transport by wind or ejection caused by rain are, in our
opinion, the most probable means by which the Pimpernel Sundew seeds are being
dispersed, but studies from the field are missing so far. The spherical seeds
are approximately 400 μm in diameter and are characterized by
a surface with concave testa cells (Fig. 1c). As concave cells are very rarely found
in fresh plant material but regularly on dry seed surfaces (Barthlott &
Hunt 2000; Koch et al. 2008), the inward deflection of the outer epidermis
wall is most probably caused by water loss and shrinkage. Moreover,
epicuticular wax crystalloids of the granule and rodlet types, typified
according to Barthlott et al. (1998), are uniformly distributed on the testa.
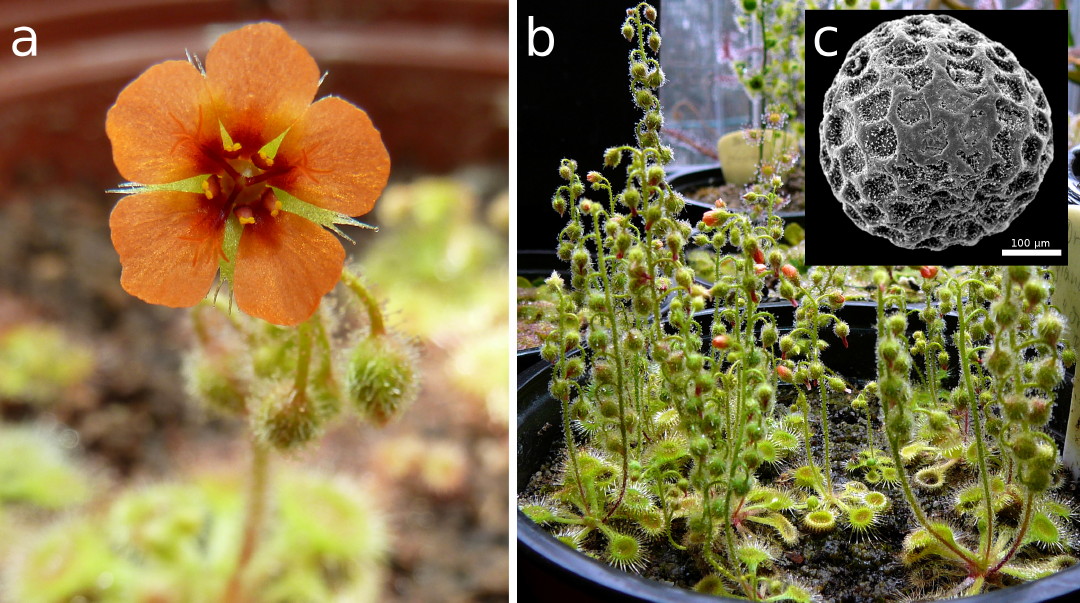 |
Figure 1: Drosera glanduligera in cultivation. a) Flower. b) Adult plants producing
numerous seed capsules on the inflorescence stalks.
c) Scanning electron
microscopy image of a seed grain featuring concave testa cells and epicuticular
wax crystalloids.
|
Prey Capture Experiments
For our prey capture experiments we
used common fruit-flies (Drosophila melanogaster). Due to their commercial
availability and easy care they were perfect specimens for our tests, although
they are unlikely to be the natural prey. The intention was to test if
snap-tentacles can fling prey animals (Davion 1995, 1999), i.e. to elucidate
their role in prey capture, under laboratory experimental conditions. It is
still up to future studies to record snap-tentacle behavior in the plant’s
habitat with natural prey (springtails, ants) and to identify its importance
for the plant. For a detailed description of “Materials and Methods” and an
outlook for future studies we refer to our original article (Poppinga et al. 2012). We placed
flies next to the plants and recorded trapping action with a HDV camcorder and
a highspeed camera with a recording speed of 2000 fps. The videos obtained
(Hartmeyer & Hartmeyer 2012a,b; Poppinga et al. 2012) clearly
show that the rapid catapult function of snap-tentacles is combined with a
slower “band-conveyor” mechanism carried out by the more centrally arranged
sticky gluetentacles. First, the prey is lifted and thrown onto the trap leaf
by snap-tentacles which, after mechanical stimulation by the animal, rapidly
bend towards the leaf center within 75 milliseconds. The prey now is in a very
disadvantageous position, because in most captures observed it was attached
with its dorsal side first to the glue-tentacles, and we hypothesize that this
mechanism also accounts for effectively immobilizing the prey. Owing to the
mechanical impact, glue-tentacles also start to bend towards the trap center,
but much slower, lasting approximately two minutes (which still is quite a fast
Drosera tentacle
movement). Hereby prey is drawn into
the deeply concave leaf-center where digestion takes place, probably well-protected
from kleptoparasites as reported from Drosera erythrorhiza (Watson et al. 1982). Unlike in
many other sundews (Darwin 1875; Lloyd 1942; Williams 1976; Juniper et al. 1989), we did not
observe leaf blade movement after capture of prey. Such a sophisticated,
combined two-step trapping mechanism is unique in the plant kingdom, and we
propose to use the term “active catapultflypaper-trap” exclusively for Drosera
glanduligera. A passive catapult-pitfall-trap system, enabled by a semi-slippery
trap surface and initiated by the impact force of raindrops, has recently been
described for the Nepenthes gracilis pitcher plant (Bauer et al. 2012),
constituting a further example of a “hybrid trapping strategy” (Rice 2007).
Tentacle Motion Analysis
How can snap-tentacles move so fast?
Active plant movements (e.g., the leaflet folding of the famous sensitive Mimosa
pudica) are often
enabled by changes in turgor pressure (cell sap pressure) in antagonistically
acting cellular tissues called pulvini (Braam 2005). Such systems are based on
a displacement of water through a porous medium, the pulvinus tissue, and hence
are actuated hydraulically. The duration of the fluid flow, and therefore the
duration of the whole movement, depends on the thickness of the tissue the
fluid has to pass. To move fast, the moving organ hence has to be small (as the
hydraulically actuated Mimosa pulvinus (Volkov et al. 2010)), or must
rely on a simple but effective “trick”: like in a bow, stored elastic energy
can be used to generate extremely fast motions “on demand” (Skotheim &
Mahadevan 2005; Dumais & Forterre 2012). For example, the Venus Flytrap features
large and fast traps (snapping lasts ~100 ms) and hence uses a buckling
instability to perform their action, comparable with a rubber-popper-toy
(Forterre et al. 2005). Other
examples are bladderworts. Although their trapdoors are quite small, their
movement is also too rapid to be actuated purely hydraulically
when performing their “ultra-fast”
opening motion in less than a millisecond (Vincent et al. 2011). The
fastest movements known in plants are achieved by explosive fracture and are
not reversible, e.g., the bursting fruits of the Sandbox Tree (Hura
crepitans) (Swaine &
Beer 1977).
Having seen the snap-tentacle bending
motion fully time resolved for the first time we also first believed that an
elastic instability is involved. More precisely, as it is a long filiform
structure that changes its curvature in short time (75 ms) we assumed a similar
mechanism as present in certain bicycle reflector bands that one strikes
against the wrist to make it curl. Here, a long, flat and relatively stiff band
with an initial transverse concave curvature of the reflector surface abruptly
switches this curvature to convex (snap-buckling) after mechanical disturbance,
which entails the fast rolling-in of the whole band (that has the intrinsic
mechanical property to curl). Surprisingly, we found that the transverse axis
of the tentacle does not undergo a sudden geometrical change, and that there
are no noticeable anatomical features (e.g., thickened cell
walls) that could take part in storing elastic energy (see also “Tentacle Morphology
and Anatomy”). As described in our original study, it can be calculated that
snap-tentacles theoretically are small enough to be actuated completely
hydraulically. Hence, we interpret the fast motion to be due to a change in turgor
pressure in antagonizing tissue layers, but further experiments, especially in
physiology, are needed for verification. As outlined in detail in the original
article (Poppinga et al. 2012), snap-tentacles function only once which is
presumably due to collapsing epidermal cells.
Tentacle Morphology and Anatomy
The spoon-shaped trap leaves of D.
glanduligera each carry a multitude of glue-tentacles, and in adult plants about
12-18 catapulting snap-tentacles that extend horizontally (in the plane of the
lamina). Mechanical stimuli on the heads of both tentacle types entail bending
motions, as described in the above section. The sticky tentacles show a bauplan
(body plan) typical for Drosera tentacles by consisting of a cylindrical stalk,
emerging almost vertically from the leaf lamina, and a more or less spherical,
mucussecreting head. The region of stalk-head-connection, where the stalk is
thinnest, is generally considered as the mechanoreceptor region (Williams
1976). Although we recorded the glue-tentacle motion, we did not investigate
their anatomy in detail. Excised snap-tentacles were analyzed with a light
microscope and a scanning electron microscope. Five μm semi-thin transverse and longitudinal sections with toluidine blue
staining were analyzed with the light microscope. For full details of materials
and methods we refer to our original article (Poppinga et al. 2012). As
detailed by Seine & Barthlott (1993), the snap-tentacles are bilaterally
symmetric. The stalk is flattened with a so-called terminal disc, somewhat
resembling a human hand, that carries the mucus-free head (Fig. 2 and Front
Cover). The flattened stalk most presumably accounts for the uniplanar, circular
bending movement the snap-tentacles perform towards the trap leaf, whereas cylindrical
glue tentacles can bend in more than one plane. The snap-tentacle’s
head-stalk-connection is very thin and most likely plays an important role in
perception of mechanical stimuli (Fig. 2). Laterally on the stalk, small
sessile glands of unknown function are visible (Fig. 3a,b). The snap-tentacle
stalk consists of outer epidermal cells, parenchymatous inner cells (Fig. 3c)
and vascular tissue. The latter consists of a tracheid system (Fig. 4a),
including a branched xylem in the head (see also Williams & Pickard 1974; Williams
1976) that is connected to a single conducting strand in the center of the
stalk (Fig. 2b,c). Epidermal and parenchymatous cells are elongated, of
variable diameters, and do not feature significantly
thickened cell walls, as already
described in the section “Tentacle Motion Analysis” (Fig. 3c). As far as we
could observe, the conducting strand in the stalk is disconnected from the leaf
lamina by ending close to the hinge-zone (Fig. 4a).
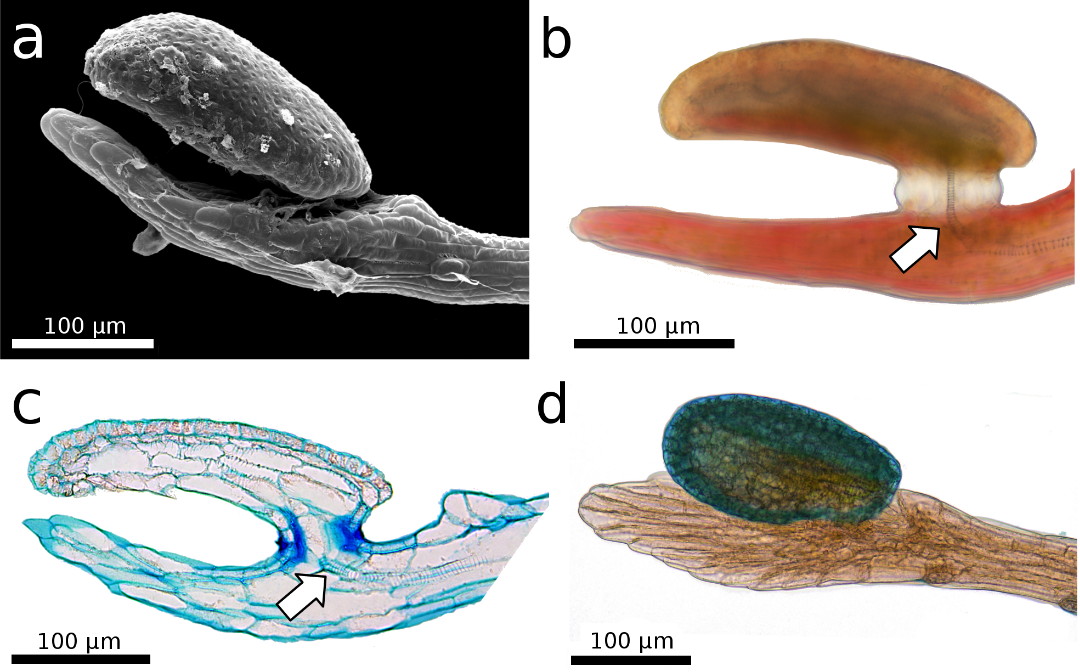 |
Figure 2: The
snap-tentacle head is raised above the stalk’s terminal disc, which somewhat
resembles a human hand. a) Scanning electron microscopy image. b,c,d) Light
microscopy images. b,c) The conducting strand is well visible (arrows). c) 5 µm
semi-thin longitudinal section, stained with toluidine blue. The thin
stalk-head-connection most presumably plays an important role in reception of
mechanical stimuli. d) The tentacle head is stained with toluidine blue.
|
The hinge zone is situated near the
tentacle base and depicts the zone where snap-tentacles bend during the fast
motion (Hartmeyer & Hartmeyer 2010; Poppinga et al. 2012).
Interestingly, here also exists a constriction with a layer of cells that
appear to be somewhat pre-cut and thereby constitute a “fault zone” (Fig. 4b).
When a slight lateral mechanical force is applied to a snap-tentacle, it will
break at this region (Fig. 4b). Although the snap-tentacle bending is extremely
fast and most presumably generates comparably high compressive and tension
forces on the cellular tissues, there exists, hence, also a mechanical weak
point (or predetermined breaking point) in this region. It can be speculated
that the conducting strand adds mechanical stiffness to the apical
snap-tentacle part, which was observed to remain straight during the motion. On
the other hand, such a strand would impede the fast bending motion of the
hinge-zone. A detailed investigation of the isolated tracheid system and of the
“breaking point” is a matter for future studies.
Transitional Stages of Tentacle
Morphology during Ontogeny
Morphological characteristics of trap
leaves and their tentacles in different ontogenetic stages ranging from
seedlings to adult plants were observed with a ProScope HR USB-microscope
(Bodelin Technologies, Oregon, USA), using a 200-fold magnification lens.
“Modern” sundew species that feature snap-tentacles typically produce two
cotyledons which are only exceptionally carnivorous, as in D. ultramafica
where they
possess a few sticky tentacles (pers. observ.). Additionally to the typical
gluetentacles on the lamina, all first trap leaves of these species possess
three to five elongated, mucus-free and bilaterally symmetrical snap-tentacles
that extend in the plane of the lamina. Hence, all these seedlings possess
fully developed snap-tentacles from the very beginning, which are able to bend
(most presumably by turgor-movement) in a time range between approximately five
seconds to a few minutes, depending on the species and the surrounding
temperature. The appearance of different snap-tentacles in the genus Drosera has been examined
during the last decade in more than 100 different species (Hartmeyer &
Hartmeyer 2010).
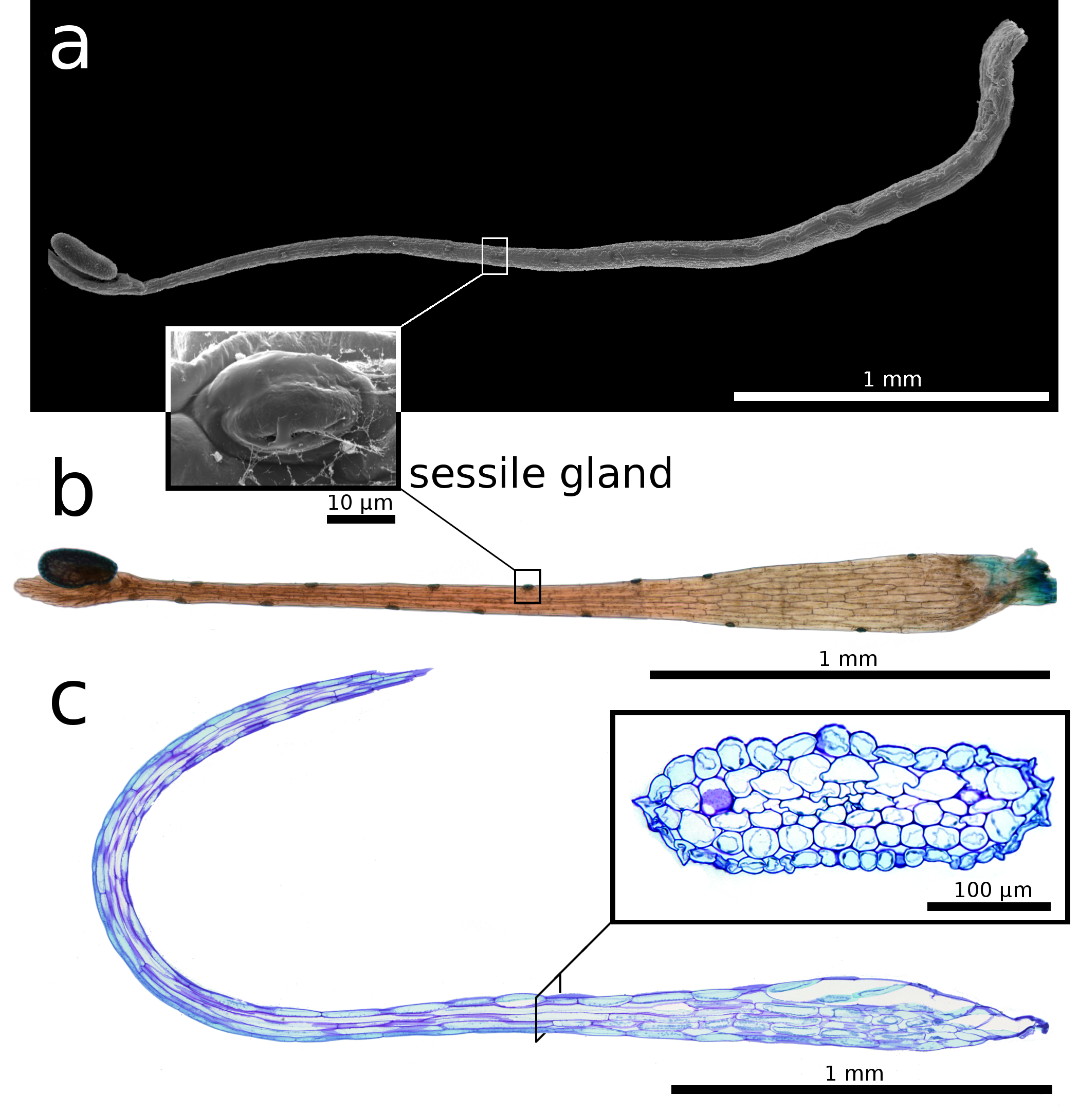 |
Figure 3: Snap-tentacle morphology and anatomy.
a) Scanning electron microscopy image. b) Light microscopy image. a, b) Sessile
glands on the stalk. c) 5 µm semi-thin longitudinal section, the insert shows a
transverse section, of the snap-tentacle stalk, both stained with toluidine
blue. |
Drosera glanduligera seeds germinate
without developing visible cotyledons; the first leaf already is a fully
functional sticky trap growing in an upright direction from the seed, but
without any snap-tentacles. The following three to four leaf generations show intermediate developmental
stages during which the elongated marginal tentacles significantly change their
morphology. The more or less spherical (symmetric), mucus-producing head
becomes replaced by the glue-free, bilaterally symmetric, raised head as
described above. As the hinge-zone of the stalk becomes more and more
pronounced, the continuous conducting strand (Fig. 5) becomes disconnected from
the lamina during the transitional stages just at the hinge region (Fig. 4).
Also, the cavity for digestion in the center of the leaf becomes more and more
distinctive. In cultivation, the first fully functional combined
catapult-flypaper-traps emerge about six weeks after germination. Although the
first catapulting tentacles still look
very tender, they are nevertheless already capable of flinging springtails
effectively onto the leaf-center (pers. observ.).
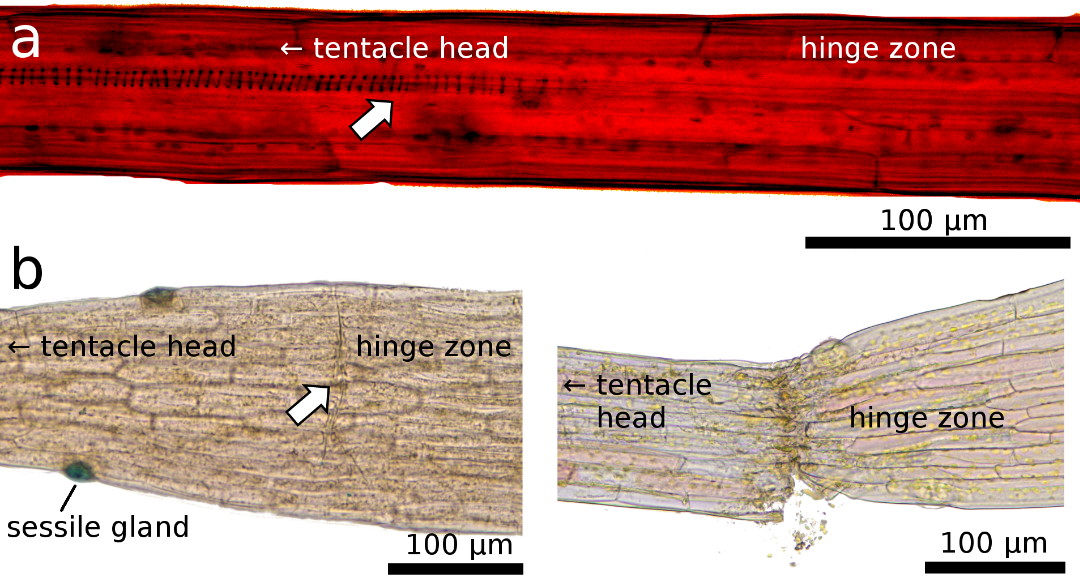 |
Figure 4: Light microscopy images of the transition zone
close to the hinge region, which is characterized by a) a disconnected conducting strand (arrow, for better visibility
structures here were enhanced by adjustment of brightness and contrast), and b) by a predetermined breaking region
with a layer of cells that appear as a pre-cut “fault zone” (arrow) that leads
to tentacle rupture when a lateral force is applied.
|
Discussion
A raised tentacle head, a disconnected
conducting strand and a preformed tentacle “breaking region”, a unique
hinge-zone, and a rapid motion comparable with the trapping speeds of Aldrovanda
and Dionaea (Ashida 1934;
Forterre et al. 2005; Poppinga
& Joyeux 2011) distinguish the catapulting snap-tentacles of D.
glanduligera clearly from all other (much slower) snap-tentacles found in other sundew
species, such as D. burmannii (Hartmeyer & Hartmeyer 2010) or D.
rotundifolia (Darwin 1875). The combined catapult-flypaper-trap comprises a
combination of 12-18 one-shot devices (the marginal
catapults) with a subsequent
“band-conveyor” consisting of sticky tentacles. Glue-tentacles are both able to
draw larger prey into the center, and to return to their initial position after
the delivery of the prey in order to wait for the next victim becoming
catapulted by snap-tentacles. We hypothesize that catapulting snap-tentacles
enable successful capture (and retention) of comparably large prey animals that
otherwise could escape from glue-only traps.
Figure 5: Digitally enlarged USB-microscope image of a
glue-tentacle in an early stage of D.
glanduligera development, which possess an obviously fully functional conducting
strand. They later become replaced by catapult-snap-tentacles in which the strand
is disconnected (see Fig. 4).
|
We hypothesize that the capture of
larger prey animals occurs only occasionally and that the capture of smaller
animals, such as springtails, being probably the main prey animals (see also
“Cultivation of Drosera glanduligera”), occurs much more frequently. Collembola
are almost
ubiquitous and find ideal life conditions in leaf litter (Fjellberg 1998),
which most probably is also true for the prey species Hypogastrura
vernalis identified by
Watson et al. (1982).
Additionally, Collembola
are reported to be caught in high
numbers by other co-occurring Australian Drosera species (Watson et al. 1982; Verbeek
& Boasson 1993). These micro-arthropods might well be attracted by chemical
volatiles that come along with wilting and wilted leaves, and we hypothesize
that this might be the case not only for D. glanduligera, but also for
many other carnivorous plants. Especially in perennial species growing as
ground rosettes, the accumulation of dead plant material could facilitate an effective attraction.
The Pimpernel Sundew has perfected its traps by the outstretched catapulting
tentacles. Another speculation is raised by the question about the function of
the sessile glands on the snap-tentacle stalks (Fig. 3a,b; 4b). Perhaps these
glands also take an active part in prey attraction by emitting scents.
Recently, attraction by sex-specific volatiles was reported for moss where the
allured micro-arthropods act as sperm dispersers (Rosenstiel et al. 2012). This
finding highlights how Collembola and other arthropod groups might be
chemically attracted. Perhaps, such mechanisms have evolved independently more
frequently and for more different purposes in plants than thought before.
|
References
Ashida, J. 1934. Studies on the leaf
movement of Aldrovanda
vesiculosa L. I. Process and
mechanism
of the movement. Mem. Coll. Sci. Kyoto
Imp. Univ. Ser. B 9: 141-244.
Barthlott, W., Neinhuis, C., Cutler,
D., Ditsch, F., Meusel, I., Theisen, I., and Wilhelmi, H. 1998.
Classification and
terminology of plant epicuticular waxes. Bot. J. Linn. Soc. 126: 237-260.
Barthlott, W., and Hunt, D. 2000.
Seed-diversity in Cactaceae subfam. Cactoideae. In: Succulent Plant
Research Vol. 5, ed. D. Hundt. David
Hunt: Milborne Port.
Bauer,
U., Di Giusto, B., Skepper, J., Grafe, T.U., and Federle, W. 2012. With a flick of
the lid: a novel
trapping mechanism in Nepenthes
gracilis pitcher plants. PLoS
ONE 7(6): e38951.
Bourke, G., and Nunn, R. 2012.
Australian Carnivorous Plants. Redfern Natural History Productions, Poole, Dorset.
Braam, J. 2005. In touch: plant
responses to mechanical stimuli. New Phytol. 165: 373-389.
Darwin, C. 1875. Insectivorous Plants.
John Murray, London.
Davion, R. 1995. Now you see it - Now
you don’t. Flytrap News 8: 17.
Davion, R. 1999. That damned elusive
Pimpernel. Flytrap News 13: 10.
Dumais, J., and Forterre, Y. 2012.
“Vegetable dynamicks”: the role of water in plant movements. Annu.
Rev. Fluid Mech. 44: 453-478.
Erickson, R. 1968. Plants of Prey.
Lamb Paterson, Perth.
Fjellberg, A. 1998. The Collembola of
Fennoscandia and Denmark Part 1: Poduromorpha. Brill,
Leiden. Forterre, Y., Skotheim, J.M., Dumais,
J., and Mahadevan, L. 2005. How the Venus Flytrap snaps. Nature
433: 421-425.
Gibson, T.C., and Waller, D.M. 2009.
Evolving Darwin’s ‘most wonderful’ plant: ecological steps to a snap-trap. New Phytol. 183: 575-587.
Hartmeyer, I., and Hartmeyer, S.R.H. 2005. Drosera glanduligera – Der Sonnentau mit “Klapp-Tentakeln”. Das Taublatt 2005/2: 34-38.
Hartmeyer, I., and Hartmeyer, S.R.H.
2006. Drosera: Snap-Tentacles
and Runway Lights. DVD documentary,
Hunting
Veggies, Weil am Rhein. Also http://www.youtube.com/watch?v=BY7z15f3Vwg
uploaded by ICPStv on Apr 16, 2010.
Hartmeyer, I., and Hartmeyer, S.R.H.
2010. Snap-tentacles and runway lights. Carniv. Pl. Newslett. 39:
101-113.
Hartmeyer, I., and Hartmeyer, S.R.H.
2012a. The Catapult-Flypaper-Trap / Die Katapult-Leimfalle
(Video:
Das Katapult der Diva). DVD documentary, Hunting Veggies, Weil am Rhein.
Also http://www.youtube.com/watch?v=Zzi3XDQs-i0
uploaded Sep. 26, 2012 by S.R.H. Hartmeyer.
Hartmeyer, I., and Hartmeyer, S.R.H.
2012b. Catapult-Flypaper-Trap: Prey-Catching Digest / Katapult-Leimfalle:
Beutefang Kurzfilm . Hunting Veggies, Weil am Rhein.
http://www.youtube.com/watch?v=eFShLcxNswk published Nov. 13,
2012 by S.R.H. Hartmeyer.
Juniper, B.E., Robins, R.J., and Joel,
D.M. 1989. The Carnivorous Plants. Academic Press, London.
Koch, K., Bhushan, B., and Barthlott,
W. 2008. Diversity of structure, morphology and wetting of plant
surfaces.
Soft Matter 4: 1943-1963.
Lehmann,
J.G.C. 1844. Nov. Stirp. Pug. 8: 37.
Lloyd, F.E. 1942. The Carnivorous
Plants. Chronica Botanica, Waltham.
Lowrie, A. 1989. Carnivorous Plants of
Australia Vol. 2. University of Western Australia Press, Perth.
McPherson, S. 2008. Glistening
Carnivores - The Sticky-leaved Insect-Eating Plants. Redfern Natural
History Productions, Poole, Dorset.
Poppinga, S., and Joyeux, M. 2011.
Different mechanics of snap-trapping in the two closely related
carnivorous plants Dionaea
muscipula and Aldrovanda
vesiculosa. Phys. Rev. E
84: 041928.
Poppinga, S., Hartmeyer, S.R.H.,
Seidel, R., Masselter, T., Hartmeyer, I., and Speck, T. 2012. Catapulting
tentacles in a sticky carnivorous
plant. PLoS ONE 7(9): e45735.
Rosenstiel, T.N., Shortlidge, E.E.,
Melnychenko, A.N., Pankow, J.F., and Eppley, S. 2012. Sex-specific
volatile compounds influence
microarthropod-mediated fertilization of moss. Nature 489: 431-433.
Rice, B. 2007. Carnivorous plants with
hybrid trapping strategies. Carniv. Pl. Newslett. 36: 23-27.
Seine, R., and Barthlott, W. 1993. On
the morphology of trichomes and tentacles of Droseraceae Salisb.
Beitr.
Biol. Pflanzen 67: 345-366.
Skotheim,
J.M., and Mahadevan, L. 2005. Physical limits and design principles for plant and
fungal
movements. Science 308: 1308-1310.
Swaine, M.D., and Beer, T. 1977.
Explosive seed dispersal in Hura crepitans L. (Euphorbiaceae). New
Phytol. 78: 695-708.
Verbeek, N.A.M., and Boasson, R. 1993.
Relationship between types of prey captured and growth form
in Drosera in southwestern
Australia. Aust. J. Ecol. 18: 203-207.
Vincent, O., Weißkopf, C., Poppinga,
S., Masselter, T., Speck, T., Joyeux, M., Quilliet, C., and Marmottant,
P. 2011. Ultra-fast underwater suction
traps. Proc. R. Soc. B 278: 2909-2914.
Volkov, A.G., Foster, J.C., Ashby,
T.A., Walker, R.K., Johnson, J.A., and Markin, V.S. 2010. Mimosa pudica: Electrical and
mechanical stimulation of plant movements. Plant Cell Environ. 33: 163-173.
Watson, A.P., Matthiessen, J.N., and
Springett, B.P. 1982. Arthropod associates and macronutrient status
of the red-ink sundew (Drosera
erythrorhiza Lindl.). Aust. J. Ecol. 7: 13-22.
Williams, S.E., and Pickard, B.G.
1974. Connections and barriers between cells of Drosera tentacles in
relation to their electrophysiology.
Planta 116: 1-16.
Williams, S.E. 1976. Comparative
sensory physiology of the Droseraceae - The evolution of a plant
sensory
system. Proc. Am. Philos. Soc. 120: 187-204.
|